The story of how a ubiquitous soil bacterium, Agrobacterium, went from being merely another plant pathogen to a household name in plant genetic engineering and plant molecular genetics serves as a case study of how basic research can lead to totally unforeseen consequences. In this narrative, I will summarize some of the landmark papers and observations which provided new insights into host-parasite interactions and contributed to this microorganism revolutionizing agriculture. Because of space limitations, many important papers and significant contributions will not be covered, and I apologize in advance for these omissions. Suffice it to say that many small groups of widely dispersed scientists working in a variety of disciplines were responsible for this remarkable tale that continues to unfold.
Early History
Fig. 1. Crown gall on Euonymus caused by Agrobacterium tumefaciens (courtesy R. L. Forster). |
|
The story begins almost exactly 100 years ago when two USDA plant pathologists, Erwin Smith and Charles Townsend published a paper in Science (1907) reporting that a bacterium that they named Bacterium tumefaciens caused plant tumors or crown galls on a variety of plants. This report was only some 20 years after the first discovery of plant pathogenic bacteria. The next major discoveries on Agrobacterium and crown gall, starting in the 1940s, were made at the Rockefeller Institute by Armin Braun whose interests in the system were initially sparked by a surprising observation of a student studying crown gall in Germany. The student found it very difficult to isolate Agrobacterium from tumor tissue. On this basis, Braun reasoned that viable bacteria might not be required for tumor growth, quite a heretical thought at the time. However, as a Post Doctoral Fellow in the laboratory of L.O. Kunkel at the Rockefeller Institute, Braun proved that this in fact is the case. He studied "secondary tumors," which develop at some distance from the site of Agrobacterium inoculation. Braun showed that they are bacteria free! From these and subsequent studies, Braun developed the concept that has guided so much subsequent research on Agrobacterium namely, a "tumor inducing principle" or TIP is responsible for tumor development. Using temperature shift experiments, he demonstrated that the TIP must be elaborated during the first 10 hours after plant inoculation. Heating inoculated plants to 32°C before this time, a temperature which does not kill either the bacteria or the plant, prevented tumor formation. After 10 hours, heating did not affect tumor formation. Braun also discovered that Agrobacterium transforms plant cells permanently in that tumor cells can grow in vitro indefinitely on medium that does not support the growth of normal plant tissue. In a remarkably prescient statement made in 1947, Braun suggested that DNA was a possible candidate for TIP, a reasonable suggestion likely made in part because colleagues at the Rockefeller Institute had recently shown that DNA could genetically transform the pneumococcus bacterium.
|
Fig. 2. Crown gall of Mark apple rootstock (courtesy A. L. Jones). |
|
|
 |
|
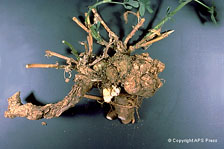 |
|
|
Fig. 3. Small, pimple-like galls of crown gall (Agrobacterium vitis) extending up the trunk of a grapevine (courtesy T. G. Burr). |
|
Fig. 4. Crown gall on a 4-year-old alfalfa plant (courtesy D. C. Erwin). |
|
To identify the TIP became the Holy Grail in crown gall research from the time that Braun postulated its existence and importance in 1947. Three publications in particular strongly pointed to DNA. The first paper, published in 1969, came from Australia where Allen Kerr demonstrated unequivocally that the property of pathogenicity could be transferred from a tumor producing pathogenic strain to a non-pathogenic strain when the latter was inoculated onto a tumor. Kerr realized that this transfer was most likely mediated by a plasmid. However, all his attempts to separate plasmid from chromosomal DNA were unsuccessful. This was not achieved for another six years.
The second publication came from the laboratory of George Morel and Jacques Tempé in France. Morel was a skilled analytical chemist who became intrigued with the crown gall problem after numerous meetings with Armin Braun. Morel’s laboratory demonstrated that crown gall tissue contained an unusual guanidine derivative, octopine, not found in normal plant tissue. This observation was clouded initially because reports from several other laboratories reported that octopine could be detected in untransformed tissues. However, for different reasons, these reports turned out to be incorrect. In the course of Morel’s studies, another guanidine derivative, nopaline, was found in a different tumor line. These guanidine compounds are given the general name of opines. Of special significance, was Morel’s group demonstration that the kind of opine found in a particular tumor depended on the strain of Agrobacterium that induced the tumor and not on the host plant. Further, this same group showed that the strain of Agrobacterium that induced tumors that synthesized a particular opine had the ability to degrade it. Tempé suggested that the same enzyme functioned in opposite directions in the tumor and in the bacteria. We now know that the synthesis and degradation are carried out by two distinct enzymes. However, the fact that tumor tissue synthesizes a compound that normal tissue does not suggested that Agrobacterium was transferring DNA that encoded an enzyme of opine synthesis.
The third intriguing observation which suggested that DNA is the TIP came from the laboratory of Robert Hamilton in the United States. From hearing a presentation by the Lippincotts, a husband and wife team studying Agrobacterium, Hamilton was aware that heating inoculated bean leaves to elevated temperatures (36°C) reduced the number of tumors. To determine if this effect was on the bacteria, he incubated Agrobacterium strain C58 on nutrient agar for 36-120 hours at 36°C. After 120 hours incubation, all of the colonies were avirulent. Hamilton suggested that a virulence factor was lost in heating. In 1970, it was known that heating bacteria at elevated temperatures could cure them of their plasmids. Hamilton and his collaborator Chris Pootjes looked for plasmid DNA in cesium chloride gradients. Although they separated chromosomal from plasmid DNA, both virulent and avirulent strains of C58 contained extrachromosomal DNA. We now know that strain C58 has two plasmids, and only the one required for virulence is eliminated by heating.
Era of Molecular Analyses Begins
With these independent observations from investigators on three different continents, renewed attention focused on DNA as the TIP. However, gene transfer between a prokaryote and eukaryote in nature had never been seen before and how prokaryotic DNA could be stably maintained and expressed in a eukaryotic cell were all questions for the future. Although many investigators were convinced that Agrobacterium must be transforming plants by transferring DNA similar to bacterial transformation, skeptics also abounded. How to demonstrate DNA transfer was a major challenge. Fortunately, the tools of molecular biology important for the handling and analysis of nucleic acids were starting to be used in the mid-1970s. Initially, these tools were often cumbersome and techniques in common use today were unavailable. Very few restriction enzymes had reached the market. Plasmid purification, especially of large plasmids, was still an art and DNA was sized in a model E ultracentrifuge. Small volumes were measured in 5-100 ul glass capillaries and PCR had not yet been invented. Kits for manipulating nucleic acids had not yet made their appearance.
Search for the TIP
Initially, the most favored candidate for the TIP was the temperate bacteriophage PS8, found in many strains of Agrobacterium. This possibility was attractive for several reasons. It was well known that viruses could transform animal cells. Further, PS8 was reported to be present in axenically grown crown gall tumor tissue. The idea gained further support from the studies of Rob Schilperoort, a pioneer in plant molecular biology at Leiden State University in The Netherlands. Schilperoort hybridized radioactive PS8 cRNA to filter bound DNA isolated from either transformed or untransformed tissue. He reported that PS8 DNA was present in transformed but not untransformed tissue. However, he later showed that the hybridization he observed resulted from non-specific binding of the RNA to impurities in the filter bound DNA. Nevertheless, it was this pioneering study that began the modern era of research on Agrobacterium.
The key paper that opened the door to the identification of the TIP came from the laboratory of Jeff Schell and Marc Van Montagu in Ghent, Belgium. This was the first of many highly significant papers from their laboratory. In 1974, they reported that eleven pathogenic strains of Agrobacterium contained large plasmids (approximately 0.2 Mb) whereas eight non-pathogenic strains did not. This correlation of plasmids with pathogenicity suggested that a large plasmid is closely related to, or maybe is even, the TIP. Direct proof that a large plasmid is important in tumor formation was not long in coming. In part, research progressed very rapidly because of an intense but fruitful competition that developed between the Ghent group ( J. Schell and M. Van Montagu) and our Seattle group (E. Nester, M. P. Gordon, and M.-D. Chilton). In 1974 and 1975, the Ghent and Seattle groups and their collaborators independently reported that strain C58 lost a large plasmid when grown at 37°C resulting in a loss of pathogenicity. Further, the cured strain regained pathogenicity when the large plasmid was reintroduced. Taken together, these data proved that a large plasmid, now labeled the tumor inducing or Ti plasmid, is required for pathogenicity. Parenthetically, the Seattle, as well as other groups had searched for plasmids unsuccessfully in Agrobacterium prior to the report of the Ghent group. However their techniques for plasmid isolation, although appropriate for isolating small size plasmids, were harsh and most likely sheared the Ti megaplasmid.
Role of the Ti Plasmid
The discovery of the importance of the Ti plasmid in crown gall raised the next obvious question, what is its role in tumor formation? The stable properties of tumor tissue, suggested that the plasmid, or some part of it, was stably maintained in the plant cell. Thus, it became critical to demonstrate that plasmid DNA was, in fact, actually present in tumor tissue. The Seattle group had previously searched for bacterial DNA and then for the entire Ti plasmid in tumor tissue without success. At this time, Southern hybridization was not yet developed. The laborious technique they used was DNA renaturation kinetic analysis in which the rate of association of a small amount of ³²P labeled single stranded DNA (probe DNA) into a double stranded form is measured in the presence of a large amount of either tumor or normal plant tissue DNA (driver DNA). If the tumor DNA contained DNA homologous to the probe, the tumor DNA would accelerate the association of the single stranded probe DNA. However, the drawback to this method is that it can detect foreign DNA only if it constitutes a significant fraction of the labeled probe. Thus, if only a few genes of the megaplasmid were present per tumor cell, they would not be detected. Since the Seattle group did not detect the entire plasmid, they were betting that only a small part was in tumor cells. They mounted a brute force effort to demonstrate this. Since it was likely that tumor tissue contained both transformed and untransformed cells, as a first step, Milt Gordon went to George Melcher’s lab in Germany where he cloned a tumor line. The renaturation kinetic analysis became an assembly line operation, with different members of the lab playing specific roles. As soon as the ³²P-d CTP arrived, a nick translation reaction was initiated to label the plasmid to its maximum specific activity. The labeled plasmid was then digested with the restriction enzyme SmaI, which we had to purify since it was not commercially available. The fragments were separated by preparative gel electrophoresis and the 15 resolvable bands were electroeluted from the gel slices. Seventy five renaturation kinetic assays were set up with labeled probes and denatured driver DNAs from either tumor or normal tissue. A total of 525 samples were taken around the clock and the percentage of single stranded probe that had renatured to double stranded DNA at each time point was determined. These experiments gave the hoped-for result. Two labeled bands renatured faster when incubated with tumor, but not normal plant DNA. No other bands did. Thus, we concluded that a fragment of the Ti plasmid was indeed present in tumor cells. The suspicions of many investigators had proven correct! DNA, and more specifically a fragment of the Ti plasmid, now named T-DNA for transferred DNA, was the long-sought-after TIP.
Integration and Transcription of T-DNA
To determine how T-DNA is maintained in the host cell genome, Mike Thomashow in Seattle analyzed four cloned tumor lines, using restriction enzymes and molecular hybridization techniques. Fortunately, by this time Southern hybridization was a proven and reliable technique. Following digestion of tumor DNA with restriction enzymes that cleaved within or outside the T-DNA and by determining the sizes and numbers of the resulting T-DNA molecules by Southern hybridization, he concluded (1) that the T-DNA is integrated into plant DNA, (2) that preferred regions of the Ti plasmid serve as points of attachment to the plant DNA and (3) that the T-DNA can be linked to more than one site in plant DNA. Later studies by Narendra Yadav in Mary-Dell Chilton’s group demonstrated that T-DNA is flanked by 25 bp direct repeats. Presumably any DNA bounded by these sequences would be transferred to the plant. Studies from several laboratories, using Southern hybridization on fractionated cell components demonstrated that T-DNA is integrated only into nuclear DNA.
The question of whether the integrated T-DNA is transcribed was quickly answered by Martin Drummond working with Mary-Dell Chilton. They demonstrated that RNA isolated from a cloned tumor line labeled with ³²P in vivo hybridized to T-DNA. Interestingly, T-DNA is not transcribed in Agrobacterium.
Coding Properties of T-DNA
Although the T-DNA with its two flanking border sequences had now been identified, how it induced crown gall tumors and what other genes are important in plant cell transformation awaited discovery. The Ghent, Leiden, and Seattle groups were the primary contributors to this part of the story. In Ghent, Marcel Holsters led the effort to determine the functional organization of the Ti plasmid of strain C58. Using transposons Tn1 and Tn7, she isolated insertion and deletion mutants and described their oncogenic and other biological properties. These studies, as well as those from the two other groups, revealed that another region of the Ti plasmid besides the T-DNA is essential for oncogenesis. Insertion of transposons in this region, now termed the virulence or vir region, created an avirulent phenotype. They also identified regions in the T-DNA and elsewhere on the plasmid that are not essential for oncogenicity.
By mutagenizing the entire genome, Dave Garfinkel in Seattle uncovered a number of loci in the chromosome that are also important for virulence. They included loci encoding proteins required for binding of Agrobacterium to plant cells, a sugar binding protein required for maximum vir gene induction, and loci required for sensing the acidic environment required for vir gene induction. Garfinkel also used site directed mutagenesis with Tn5 to develop a fine structure map of the T-DNA in order to identify the function of genes responsible for tumor formation. He, together with several colleagues in Seattle and Gert Ooms, a visiting graduate student from Rob Schilperoort’s lab, isolated 75 Tn5 and 3 Tn3 insertions which identified four genetic loci. Tms mutants (tumor morphology shooty) resulted in shoot proliferation in the tumor; tmr (tumor morphology rooty) resulted in root formation and insertions in tml (tumor morphology large) gave rise to unusually large tumors on certain plants. Gert Ooms made similar observations independently. Later studies showed that the "shooty" mutations resulted from insertions in two loci (iaaH and iaaM) concerned with auxin synthesis and the "rooty" mutations contained an insertion in a gene for cytokinin synthesis (ipt). Interestingly, no single insertion resulted in avirulence. In later studies, Walt Ream showed that an avirulent phenotype required simultaneous insertions in both genes coding for auxin and cytokinin biosynthesis.
Transfer of T-DNA
Once T-DNA was identified and characterized, attention turned to the question of how does Agrobacterium transfer T-DNA into plant cells. The vir region of the Ti plasmid is highly conserved in octopine and nopaline strains and was surmised to be involved in the transfer process. As a first step to constructing a fine structure map of this region, Scott Stachel and Gyn An in Seattle constructed a Tn3 lacZ transposon which could randomly generate gene fusions as well as serve as a reporter to study gene expression. They identified six complementation groups in this region. In the course of these studies, Stachel also observed that expression of the vir genes was enhanced significantly by exudates of a variety of plant cell cultures. From Seattle, Stachel went to Ghent where he and his collaborators then identified two phenolic compounds, acetosyringone (AS) and hydroxyacetosyringone (OH-AS) in plant exudates that induce the vir genes.
Stachel was also an important player in elucidating the mechanism by which AS induces the vir genes. He and Steve Winans in Seattle independently characterized a two-component system, VirA/G which controls expression of the vir regulon. AS binds to the membrane bound histidine kinase sensor protein, VirA which then activates the response regulator VirG, which in turn binds to upstream regions of each of the vir operons and activates their transcription. This particular two-component regulatory system was one of the very first to be described in any organism.
Stachel and Pat Zambryski also demonstrated that the addition of AS to Agrobacterium results in the formation of a single stranded, linear DNA molecule, which they suggested is the intermediate in the transfer of T-DNA to plant cells. They further suggested that this form of DNA, now termed the T-strand resulted from an endonucleolytic cleavage at the right and left borders of the T-DNA and compared the transfer of this DNA to transfer of single stranded DNA in bacterial conjugation.
The publication of Stachel et al was quickly followed by a paper by Martin Yanofsky et al in Seattle which demonstrated that the virD operon encodes a site-specific endonuclease that cleaves within each of the 25 bp direct repeats that flank the T-DNA. Two proteins are required, Vir D1 and Vir D2. Once the Vir D2 protein cleaves the DNA it remains covalently attached to the 5′ end of the T-strand, as first demonstrated by Calvin Young et al. This protein likely serves as a pilot protein for entry of the T-DNA into the plant cell nucleus. In support of this idea, Luis Herrera-Estrella in Ghent demonstrated that the amino-terminal portion of the Vir D2 protein contains a nuclear localization signal. Another Vir protein, Vir E2 was shown by Barbara Hohn et al in Basel, Switzerland to be a single stranded DNA binding protein which also has nuclear localization signals. This protein not only protects the T-strand from degradation by plant nucleases but also helps direct the T-strand into the plant cell nucleus.
One of the most challenging questions of this interkingdom transfer of DNA is how is the T-DNA transferred from bacteria to recipient cells. This problem is being studied in numerous laboratories but the person who has made especially significant contributions is Peter Christie at the University of Texas. The eleven open reading frames of the vir B operon encode a trans envelope protein complex as well as the T-pilus. The various proteins which make up this organelle provide the channel as well as the energy for the transfer of the T-strand. Another protein, Vir D4, is a coupling protein which recruits the effector proteins to the transenvelope protein complex. Thus, it is likely that the transmembrane protein complex, the T-pilus and the coupling protein function as a single organelle to translocate the T-strand as well as several other Vir proteins into host cells. This type IV secretion system (T4SS), first identified as the conjugation apparatus in E. coli, has now been identified in many species of Gram-positive and Gram-negative bacteria; however, Agrobacterium serves as the model system. The T4SS of Agrobacterium is unusual in that both DNA and many Vir proteins are transferred. The Vir proteins, such as VirE2, VirE3, and Vir F are transferred independently of DNA, but through the same T4SS.
Hijacking of Plant Signals and Structures by Agrobacterium
Agrobacterium is a very clever organism in that it uses numerous plant metabolites and cellular structures to further its own ends. We have already discussed how Agrobacterium recognizes a wound site on a susceptible plant through the plant metabolite AS which is involved in vir gene activation. Several other examples are known. Once the T-DNA is transferred into the plant, the vir regulon no longer needs to be expressed. Therefore, one of the products of T-DNA expression, indole acetic acid (IAA), shuts down expression of the vir regulon by inhibiting the activation of the VirA/G regulatory system, thereby conserving carbon and energy. At slightly higher concentrations, IAA kills the bacteria which may help explain the long standing observation that crown gall tumors often do not contain viable bacteria.
Another product of transformed plant cells which plays an important role in the biology of Agrobacterium are the opines. Studies from the laboratories of Stephen Farrand, Steve Winans, Allen Kerr, and Max Tate demonstrated that certain opines induce the synthesis of a signal molecule, acyl homoserine lactone which interacts with a transcriptional activator to promote conjugal transfer of the Ti plasmid to other Agrobacteria.
Not only does Agrobacterium utilize extracellular plant signals in the infection process, but it also hijacks cellular processes for the transport of the T-strand to the nucleus. These pioneering studies have been carried out largely in the laboratories of Stanton Gelvin, Vitaly Citovsky, and Tsvi Tzfira. The viscous cytoplasm with its assemblage of membranous and non membranous structures most likely requires that the T-strand be actively transported to the nucleus. Many DNA viruses use dynein motor proteins of the host and the host microtubule system for their transport to the nucleus. The available data suggest this may be true for the journey of the T-strand to the nucleus also. Both Vir D2 and Vir E2 proteins with their nuclear localization signals are important in nuclear import and both accumulate in the plant cell nucleus. A number of other host proteins have been implicated in their interaction with the T-strand but the roles they play in the transformation process are obscure.
Genetic Engineering of Plants
Soon after it was shown that T-DNA was integrated into the plant cell nucleus and that the T-DNA was defined by its flanking border sequences, many laboratories realized that Agrobacterium potentially might be a very useful vector for introducing any desired DNA into plants. This possibility was strengthened when Léon Otten in Jeff Schell’s group demonstrated that opine genes were transferred in a Mendelian fashion. Self pollination of the transformed plant and crossing of transformed and untransformed plants showed that this trait was transmitted through pollen and eggs as a single dominant gene with the predicted Mendelian ratios. Jean-Pierre Hernalsteens then inserted a Tn 7 transposon into an opine locus and demonstrated that it was transferred to, and maintained in, tumor tissues induced by this mutant strain. Since Tn5 had its own promoter, antibiotic resistance was not expressed. However, this experiment did demonstrate that using the Ti plasmid as a vector system for introducing DNA into plant cells was feasible.
The major technical problem in using the Ti plasmid as a gene vector is its large size. This makes it impossible to employ a direct recombination strategy to insert genes of interest into the T-DNA. Several indirect methods were developed in the early 1980s for manipulating T-DNA but these were cumbersome and not easily carried out. The problem was solved when two independent groups, one led by Mary-Dell Chilton and the other by Rob Schilperoort, shrank the plasmid by separating its two essential regions into two plasmids; the 25 bp borders flanking the T-DNA between which genes of interest could be inserted and the vir region which is necessary for the processing and transfer of the T-DNA. This binary vector system is used in most laboratories today.
Two additional hurdles remained before plants could be routinely transformed in an industrial setting with genes that create added value on the engineered plants. First, plants had to be regenerated with the genes of interest stably maintained and second, the introduced genes had to be expressed. The first problem was solved by two independent groups Pat Zambryski et al in Jeff Schell’s group and Andy Binns et al at the University of Pennsylvania. They both showed that the oncogenes of T-DNA interfere with normal plant cell differentiation and that if they are removed, transformed cells readily regenerate into normal plants. The second hurdle was overcome when three independent groups, (the Ghent group, a group from Monsanto headed by Rob Fraley, and Chilton’s group) almost simultaneously reported on the expression of foreign genes in transformed plants. All three groups used the nopaline synthase promoter fused to an antibiotic resistance locus which resulted in antibiotic resistant tobacco transformants. These experiments opened up the age of plant genetic engineering with the Monsanto Company leading the subsequent industrial revolution in agriculture.
Although Agrobacterium has a very broad host range and tumors have been detected on many dicotyledonous plants in Nature, the most important plants economically worldwide are the monocots, which include the cereals. Since crown gall tumors had never been observed in this group of plants, and initial efforts to transform many of them in the laboratory were unsuccessful, many agrobiologists believed that monocots were totally recalcitrant to Agrobacterium transformation. However, a clever experiment performed in Barbara Hohn’s group, on the suggestion of her virologist husband, clearly demonstrated in fact that Agrobacterium was able to carry out the initial steps in maize transformation-the transfer of T-DNA and expression of the transferred genes. These investigators introduced tandemly repeated copies of the genome of maize streak virus between the T-DNA borders and inoculated maize plants with Agrobacterium carrying this construct in a binary vector. The inoculated leaves developed symptoms of maize streak viral disease! Further experiments demonstrated that this viral infection, termed agroinfection requires the same Vir proteins as are required for tumor formation by wild type Agrobacterium. These results encouraged others to test a variety of strains of Agrobacterium and different monocot tissues using a variety of reporter systems and selective markers to look for T-DNA transfer and expression. In 1994, Hei and his colleagues at the Japan Tobacco Company reported the efficient transformation of rice. They studied a variety of tissues and several different strains of Agrobacterium, screening for GUS color and selecting for hygromycin resistance. Of all the tissues tested, scutellum callus gave the highest level of transformation and a "super-binary" vector gave especially high transformation frequencies of various cultivars of Japonica rice. High frequency Agrobacterium transformation has now been achieved for most cereal crops.
Expanded Host Range
Although Agrobacterium gene transfer and crown gall tumor formation in a wide variety of plants is a common occurrence in nature, in the laboratory, Agrobacterium can transfer DNA into a much broader group of eukaryotic cells. This was first demonstrated by Paul Hooykaas and colleagues who reported that Agrobacterium carrying a ura+ locus between T-DNA borders could transform a ura- strain of the yeast Saccharomyces cerevisiae to prototrophy in medium that promoted vir gene induction. This group also showed that many other fungi could be transformed following co-cultivation with Agrobacterium. Now, numerous algae, protozoa and even Hela cells have been stably transformed in the laboratory. These reports have opened up a whole new use for Agrobacterium, allowing the genetic analysis of organisms that previously were totally recalcitrant to such studies.
T-DNA in Untransformed Plants
Agrobacterium is truly a natural genetic engineer, in that not only does it transform plants to synthesize food (opines) for itself but its DNA can be found even in perfectly normal appearing, uninfected plants. In Seattle, Frank White and colleagues reported that the T-DNA (rol) genes of A. rhizogenes can be readily detected in uninfected N. glauca and many other species of tobacco. It seems most likely that A. rhizogenes infected a Nicotiana plant millions of years ago to form hairy root tumors which then regenerated into normal appearing plants which passed down the conserved sequences to their progency. This observation puts to rest the idea that transgenic plants represent an unnatural phenomenon that only occurs in the laboratory. One can safely bet that the finding of T-DNA in many species of Nicotiana is not an isolated phenomenon limited to this plant.
Concluding Comments
Research on crown gall tumors began as a classic study in plant pathology aimed at identifying the cause of a devastating plant disease with unusual symptoms. Studies on Agrobacterium by investigators in laboratories around the world have revealed new biological phenomena and practical applications far beyond what Smith and Townsend could ever have imagined. On the one hand, Agrobacterium causes serious problems for growers of grapes, stone fruits, and ornamental plants. On the other hand, it has provided an unusually exciting and rewarding experimental system for those of us fortunate to have entered into its study. The study of Agrobacterium-plant interactions has led to many unanticipated pleasant surprises, many of which were first observed in this system but now have been found to be common in the biological world.
Many of the blockbuster discoveries on Agrobacterium-plant interaction have been cited briefly in this narrative. However, much remains to be learned. The chemical signaling between Agrobacterium and plants in the natural environment of the plant’s rhizosphere has yet to be fully explored. In addition, the trafficking of the T-strand from the inception of the transfer process to the plant cell nucleus provides an area of fruitful research opportunities for interdisciplinary investigations. The full potential of using Agrobacterium as a mutagen and a transfer system for genes into an ever expanding number of eukaryotic cells has yet to be realized. After 100 years, the tale of Agrobacterium is not yet finished.
Selected References
McCullen, C.A. and Binns, A.N. 2006. Agrobacterium tumefaciens and plant cell interactions and activities required for interkingdom macromolecular transfer. Annu. Rev. Cell Dev. Biol. 22:101-27.
Nester, E., M.P. Gordon, A. Kerr. eds. 2005. Agrobacterium tumefaciens: From Plant Pathology to Biotechnology. American Phytopathological Society, St. Paul, M.N.
Tzfira, T. and V. Citovsky. eds. 2008. Agrobacterium: From Biology to Biotechnology. Springer Science, N.Y.
Tzfira T. 2006. On tracks and locomotives: the long route of DNA to the nucleus. Trends Microbiol. 14:61-63.